- Home
- News
- Analysis
- States
- Perspective
- Videos
- Education
- Entertainment
- Elections
- World Cup 2023
- Features
- Health
- Business
- Series
- Economy Series
- Earth Day
- Kashmir’s Frozen Turbulence
- India@75
- The legend of Ramjanmabhoomi
- Liberalisation@30
- How to tame a dragon
- Celebrating biodiversity
- Farm Matters
- 50 days of solitude
- Bringing Migrants Home
- Budget 2020
- Jharkhand Votes
- The Federal Investigates
- The Federal Impact
- Vanishing Sand
- Gandhi @ 150
- Andhra Today
- Field report
- Operation Gulmarg
- Pandemic @1 Mn in India
- The Federal Year-End
- The Zero Year
- Premium
- Science
- Brand studio
- Home
- NewsNews
- Analysis
- StatesStates
- PerspectivePerspective
- VideosVideos
- Entertainment
- ElectionsElections
- Sports
- Loading...
Sports - Features
- BusinessBusiness
- Premium
- Loading...
Premium
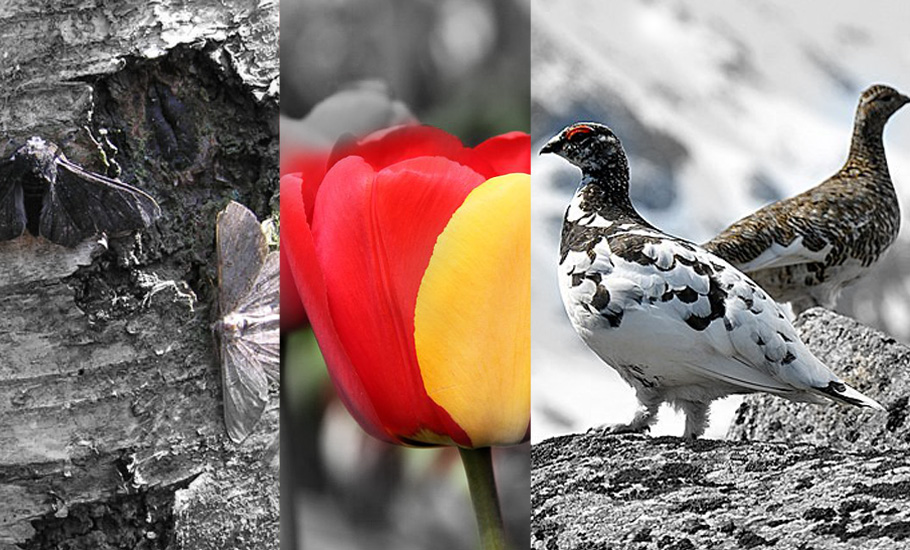
Diversity or mutation, what drives evolution?
“It takes all the running you can do, to keep in the same place.” As the Red Queen famously states to Alice in Lewis Caroll’s Through the Looking-Glass, all species must evolve to hold up. Over millennia, the river changed its course flooding a new place and aridity in what was once a lush green region. Periodically, the Earth’s orbital inclination undergoes change...
“It takes all the running you can do, to keep in the same place.”
As the Red Queen famously states to Alice in Lewis Caroll’s Through the Looking-Glass, all species must evolve to hold up. Over millennia, the river changed its course flooding a new place and aridity in what was once a lush green region. Periodically, the Earth’s orbital inclination undergoes change bringing forth ice ages and warm interglacial periods. A chance meteorite strike may destroy a habitat endangering diverse species living there. To survive, all species must evolve and adapt to the ever-changing environment or face extinction.
The process of adaptation by natural selection in a new environment can be driven more by random mutations or by the initial diversity in the gene pool. While the role of both in evolution is evident, which one is a significant driver of evolution? Pre-existing standing genetic variations or novel de novo mutations? This has been a vexing question in evolutionary biology.
By experimentally testing 72 different populations of Escherichia coli (E.coli) bacteria over 2,000 generations, Minako Izutsu and Richard E Lenski found that in the long run, surprisingly, the effect of the pre-existing variation becomes inconsequential. In contrast, the role of the novel new mutations becomes considerable. They work at the BEACON Center for the Study of Evolution in Action, Michigan State University, East Lansing, Michigan, USA. Their work is currently available as a preprint on BioRxiv ahead of peer review.
Diversity and natural selection
Around the 1840s, trapping a totally black peppered moth (Biston Betularia) near the centre of Manchester, RS Edleston, a naturalist and entomologist, was over the top. No one ever had captured a dark black peppered moth until then. The peppered moth came in various shades of grey, from light to black, yet the black ones were a rarity. However, by the 1900s, in English cities, almost all the peppered moths were dark. Naturalists began to notice and wonder about the near disappearance of light-shaded moths from cities.
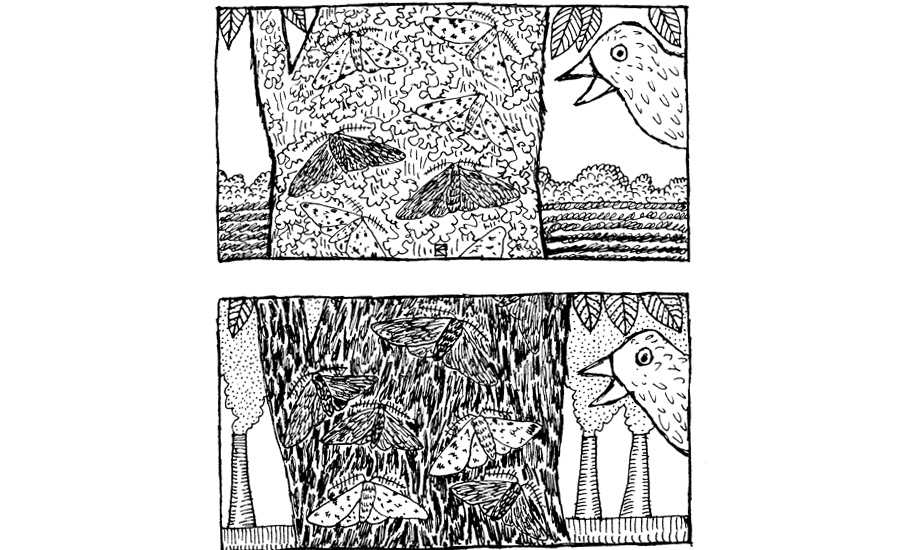
Taking a cue from Darwin, JW Tutt suggested in 1896 that the increase in the proportion of black peppered moths is the handiwork of natural selection. In the dark black background of the soot from the coal burned in the industries, settled on the trees and bushes, the light-coloured moths were an easy target for the waiting predatory birds. Black moths could camouflage and escape the birds in the dark, dusty cities and towns. With more and more light moths becoming easy prey, the proportion of the black moths slowly increased. From the late 20th century, European cities significantly reduced their pollution and are becoming cleaner. With the towns becoming greener, the number of light-coloured moths rose in good proportion, as predicted by the theory.
Life is diverse – from giant blue whales to tiny E.coli. Individuals of a species are also distinct from each other. Each of them bears a specific set of heritable traits. In the ordinary course of life, most characteristics are of no consequence. Still, when the environment changes, some heritable traits may become advantageous. Individuals of that species will have more reproductive success, resulting in more descendants with that specific trait.
For instance, in the textbook case of evolution by natural selection, the genetic allele for the ‘black’ coloured moths did not emerge de novo. It was already present in the gene pool. In a new environment, in this case, resulting from the industrial pollution, one of the traits, that is ‘black’ allele, acquired an advantage over others. Thus, on average, the population turned darker.
Novel mutation
In contrast, the novel coronavirus – that is, SARS-CoV-2, has been dodging our salvos by de novo mutations. The genetic instructions of this virus are encoded in 29,900 ‘letters’ of RNA — a, c, g and u. The virus makes a million copies of itself once inside the human cell. It is but natural that typos occur when the cell replicates the genome. Usually, just a single wrong letter is inserted in the genome. These types are called mutations. One estimate says that once in 15 days, a novel coronavirus mutates. For example, compared to the first genomic sequence, Wuhan-Hu-1 collected on December 26, 2019, in the genome WH-9 collected on January 8, 2020, the 186th letter of RNA was ‘u’ instead of ‘c’. When the virus spreads from person to person, mutations accumulate. For instance, the genome GZMU0030 collected on February 27, 2020, from Guangzhou, China, had two additional mutations making it differ at three points compared to the Wuhan-Hu-1 sequence.
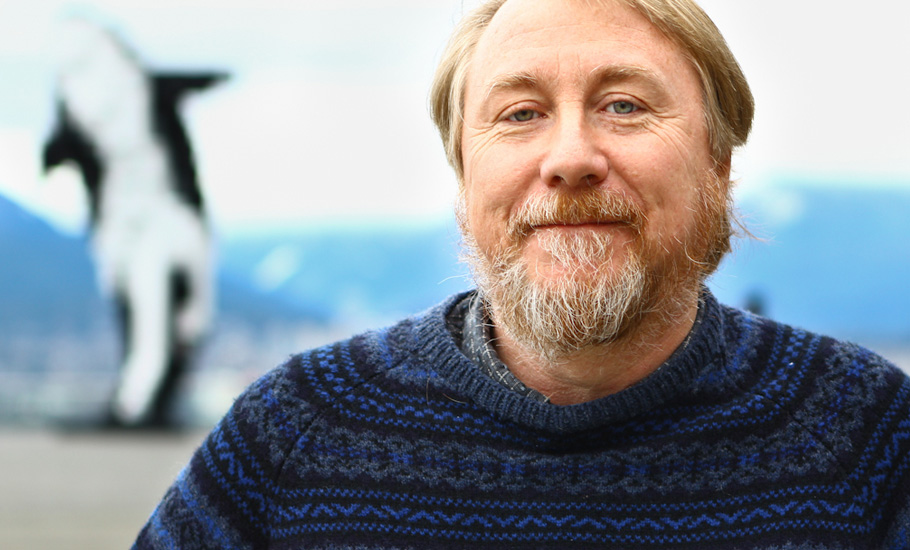
While mutations are frequent, most are insignificant and hardly make any difference to the organism. Nevertheless, the mutation may sometimes modify a crucial point in the genome, and a novel trait may emerge. For example, the chance mutation that occurred at the 1841st place in the 3818 letter-long genomes of the spike protein changed the characteristic of novel coronavirus. The letter ‘a’ changed to ‘g’ at this genomic location. As a result, in the mutant virus, the genetic instructions for the 614th amino acids switched from a “D” (short for aspartic acid) to a “G” (short for glycine). Called D614G mutant, this single typo made the virus more infectious and transmissible.
Like the spikes on the skin of the jackfruit, about 100 spikes protrude from the novel coronavirus. With spikes, the virus grabs the human ACE2 receptor on the surface of the cells lining the respiratory tract. Once spikes lock onto the ACE2 receptor, the virus can enter and cunningly use the cellular machinery to make copies of itself. Not all the spikes on the virus surface are functional. But due to the D614G mutation, more of the spikes became operative. Thus, the virus could latch on to the ACE2 receptor more efficiently than the ancestral variants. Having become better at infecting the upper airway, the variant replicated more significant numbers and turned highly transmissible in humans. Laboratory studies on hamsters confirmed that the D614G mutant had enhanced infectivity and competitive fitness when faced with the ancestral variant.
To tide over the pandemic, we took precautionary steps. With unprecedented global lockdown, masks, social distancing and hand washing, we disrupted the chain of viral transmission. Without the human host to infect and replicate, the coronavirus is a lost cause. The D614G mutant became more fit in this new environment than the ancestral variants.
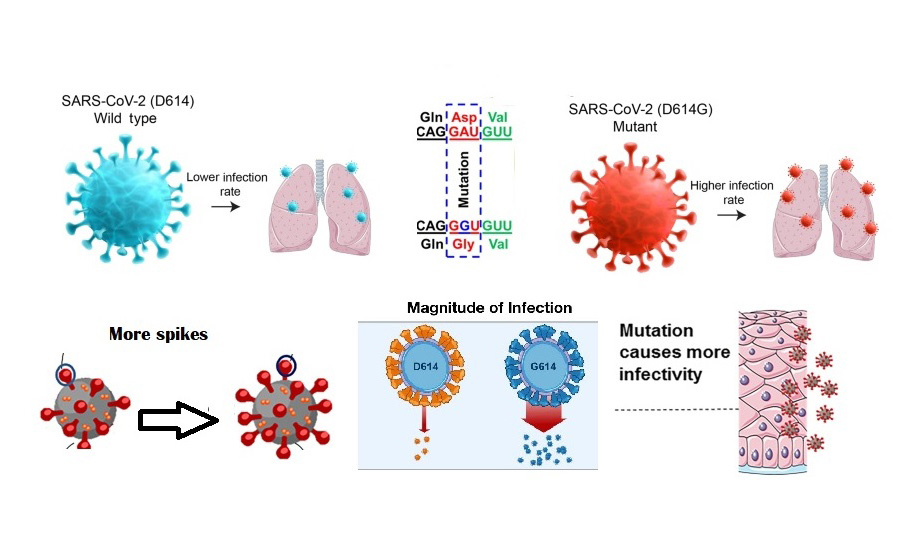
The mutation is estimated to have occurred sometime during January (10–25) 2020. By June 2020, the D614G variant had become dominant worldwide. Like the black moths replaced the rest, the de novo mutant D614G and its descendants replaced all the earlier variants. As the virus stack up advantageous mutations, they begin to improve. In the language of evolution, in other words, they become more fit. Thus fitter variants, alpha, beta and so on emerged.
The experiment
The standing genetic variation is those alleles that existed in a population when the environment started changing. The alleles that resulted in the hues of grey, from light to dark, are an example of standing genetic variation. In contrast, new, de novo mutations are those alleles introduced after that point. For instance, the D614G mutation in novel coronavirus is a unique and novel mutation that occurred after the non-pharmaceutical interventions such as masking and staying at home. It is not necessary that moth had to have had black hue to escape the dark dusty industrial English towns.
Perhaps a chance mutation might have made one variant nimble, at the blink of an eye flutter away escaping the clutches of the predators. Or else the mutation made it taste bitter, dissuading the birds. Novel mutations could have achieved what was accomplished by the initial colour variation with the population of the peppered moths. All genetic variation starts as new mutations and later becomes standing variation. The timing is essential to understanding the evolutionary dynamics between pre-existing variations and novel mutations.
The study by Izutsu and Lenski used six founder populations of E. coli, a type of bacteria found in the gastrointestinal tract of humans and warm-blooded animals. The genome of this bacteria has 46,39,221 base pairs and hence viable to keep tract of the genetic mutations over generations. At the start of the experiment, each of the 72 test tubes had a population of E. coli bacteria with various amounts of genetic diversity. As the E. coli multiplied and new generations were produced, the researchers compared the rates of adaptation in a population of E. coli with no initial diversity with populations of various levels of diversity. From this, they teased out the impact of standing genetic variation in the population and the influence of novel mutation in driving evolution. The study was part of the long-term evolution experiment (LTEE) using E. coli.
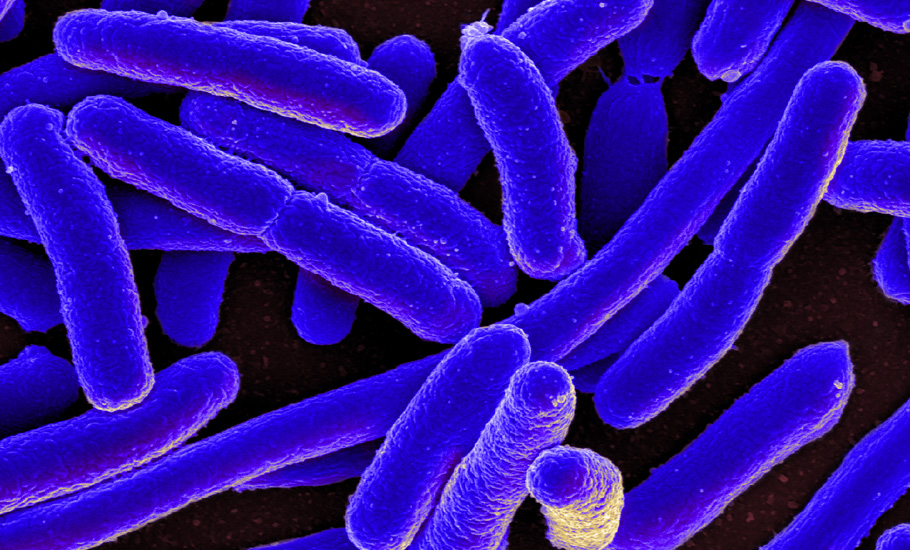
The first set of tubes had bacteria cloned from one E.coli from each of the six founder populations. That is, the cultured bacteria in the test tube were genetically identical. With no initial genetic variation, these bacteria had to rely entirely upon new novel mutations to adapt. In evolution, if the standing variation is primary, then one would expect with no initial variation, these populations in the long run will be less fit.
The next set of test tubes had bacteria cultured from one of the founder populations. In a population of bacteria, each one is not genetically identical. Thus these had all of the common genetic variations present in that population. Natural selection could act on these common alleles.
The third set of tubes had a mixed clone population. As the test tubes contained an admixture of the six founding clones, the starting diversity was only between the clone populations. However, within populations, the bacteria were genetically identical.
The fourth set of tubes had an admixture of the six founding populations harbouring all of the genetic variations in the other three treatments. Suppose the standing genetic variation is the key driver of evolution, being most diverse, this must fare far better than the other three.
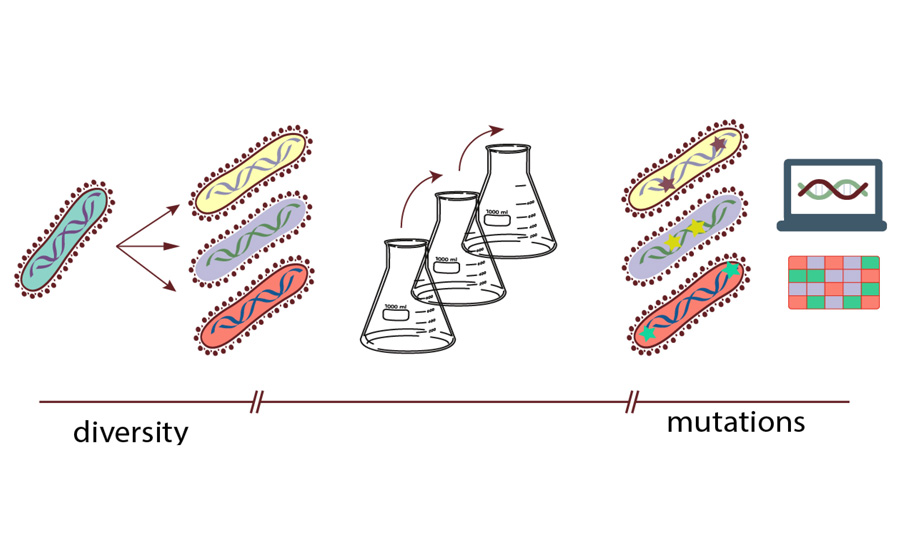
In a nutshell, at the one end of the spectrum, the test tube had bacteria without any variation, identical to each other, in the middle population of bacteria with a variation. At the far end of the spectrum, the populations varied, but there was also diversity by admixture population. To avoid random errors, the researchers prepared 18 sets of duplicates in each of the four treatments. All of the cultures were tended the same as the bacteria grew and multiplied.
Typically E.coli cultured in test tubes are fed with glucose-rich nutrient solution. Glucose provides the energy and carbon required for cell growth. Evolution by natural selection and adaptation occurs when there is a drastic change in the living environment of the organism. In the experiment, the researchers reduced the amount of glucose and added an amino acid D-serine to all the 72 test tubes to alter the growth environment and push the bacteria to evolve. In the altered growth environment, starvation and competition for food were the key challenges. In the absence of adequate glucose, each individual E.coli had to compete with each other for food. Variations within the population would vie with one another so would different populations struggle for the resource. The struggle for resources, researchers hoped, would drive evolution.
Findings
In 300 days, the E.coli population in each of the 72 test tubes underwent 2,000 generations. With each passing generation, here and there, mutations took place. Some of the mutations were bad for the bacteria. Bacteria with deleterious mutations would not leave much offspring. Hence in the next generation, their proportion in the population would diminish. Slowly and steadily, these mutations would be naturally eliminated.
On the other hand, if a mutation aids them in reproducing fast, lineage with these traits will become most abundant. Over time the beneficial novel mutations will become part of the genetic makeup of the whole population. With bacteria amassing beneficial mutations, it begins to improve and progressively become more ‘fit’. The fitness in the experiment is measured by how fast the bacteria multiplied and grew compared to a common variant of E.coli not included in the experiment. At the 0, 500, and 2,000 generation points, the researchers took sample cultures from all the 72 test tubes. They measured their ability to compete for nutrition resources.
As expected, comparatively, those with diversity had significant fitness at the start of the experiment. Those with standing variation continued to have an edge until around 100 generations. They evolved to better compete for food than those that started as less diverse. But by the time 500 generations were cultured, the initial standing genetic variation was of no consequence in any of the four experimental aggregates. In the 2000 generation, all the four observed aggregates adapted equally well, despite the variation in fitness at the start. In the long run, the initial edge due to diversity had disappeared altogether. The results suggest that random mutations are the primary driver of evolution, rather than diversity, at least in E.coli.
The lifespan of bacteria and viruses is short; each day, E.coli undergoes 6.64 generations. Hence, one can witness large enough generations within a matter of years. Therefore it is practical to undertake long-term evolution studies on bacteria. The life span of plants and animals is longer. Hence, one must wait hundreds of years to conduct experiments in plants and animals to tease out the effect of prior standing genetic variation and new novel mutations. Therefore researchers studying animals and plants often tend to stress the diversity of the gene pool as the crucial source of evolutionary capacity.
Although this experiment suggests the primacy of novel mutations over the standing genetic variations, the authors caution. Both forces “can contribute sequentially, simultaneously, and even synergistically to the process of adaptation by natural selection”, researchers write. Further the study is yet to be peer reviewed and only pre-print has been published. Sceptical review by experts could ferret out further limitations of the conclusion. Future experimental studies with complex organisms will help refine the findings.