- Home
- News
- Premium
- THE FEDERAL SPECIAL
- Analysis
- States
- Perspective
- Videos
- Sports
- Education
- Entertainment
- Elections
- Features
- Health
- Business
- Series
- Bishnoi's Men
- NEET TANGLE
- Economy Series
- Earth Day
- Kashmir’s Frozen Turbulence
- India@75
- The legend of Ramjanmabhoomi
- Liberalisation@30
- How to tame a dragon
- Celebrating biodiversity
- Farm Matters
- 50 days of solitude
- Bringing Migrants Home
- Budget 2020
- Jharkhand Votes
- The Federal Investigates
- The Federal Impact
- Vanishing Sand
- Gandhi @ 150
- Andhra Today
- Field report
- Operation Gulmarg
- Pandemic @1 Mn in India
- The Federal Year-End
- The Zero Year
- Science
- Brand studio
- Newsletter
- Elections 2024
- Events
How fast the Universe is expanding: astronomers are in a conundrum
Imagine the bewilderment if the measure of a person’s height taken from toe to head differs greatly from the value obtained by calculating from head to toe. Astronomers are currently dealing with such a predicament.The Hubble constant, a vital cosmological parameter that describes how fast the Universe expands, also sets the absolute scale, size and age of the Universe. The value arrived at...
Imagine the bewilderment if the measure of a person’s height taken from toe to head differs greatly from the value obtained by calculating from head to toe. Astronomers are currently dealing with such a predicament.
The Hubble constant, a vital cosmological parameter that describes how fast the Universe expands, also sets the absolute scale, size and age of the Universe. The value arrived at by cosmologists using the distance ladder method is markedly different from that obtained by studying Cosmic Microwave Background (CMB) Radiation, a relic of the earliest light produced in the Universe before the birth of stars. Despite our best efforts and the use of advanced technology, the discrepancy persists. In fact, with each precise measurement, the gap widens. The latest results reveal a staggering nine per cent discrepancy. This puzzle, called 'Hubble tension', indicates that we may have overlooked something crucial in the widely accepted Big Bang theory.
Expanding Universe
From theoretical considerations, Russian physicist Alexander Friedmann, in 1922, initially concluded that the Universe must be expanding. This idea was further enriched by Belgian astronomer Georges Lemaître in 1927. However, it remained a mere theoretical speculation until an American astronomer, Edwin Hubble, provided the confirming observational evidence in 1929. Using the 100-inch Hooker Telescope on Mount Wilson in California, Hubble measured the distance of 24 nearby galaxies and the speed at which they were receding into space away from us. To his surprise, he found that the farther the galaxy, the greater the speed at which it moved away from us. When Hubble plotted the observed velocities versus their distance, the graph clearly showed that the Universe was expanding uniformly in all directions. Later, he observed hundreds of galaxies and found the same results; the farther the galaxy is, the faster it recedes from us.
The idea of expanding Universe is complex. Thus, an analogy will help us understand it. Assume you attach one end of an elastic string to a hook on the wall. Now, mark evenly spaced dots along the length of the thread, one for every centimetre. Now, grasp the loose end and stretch it to double the size.
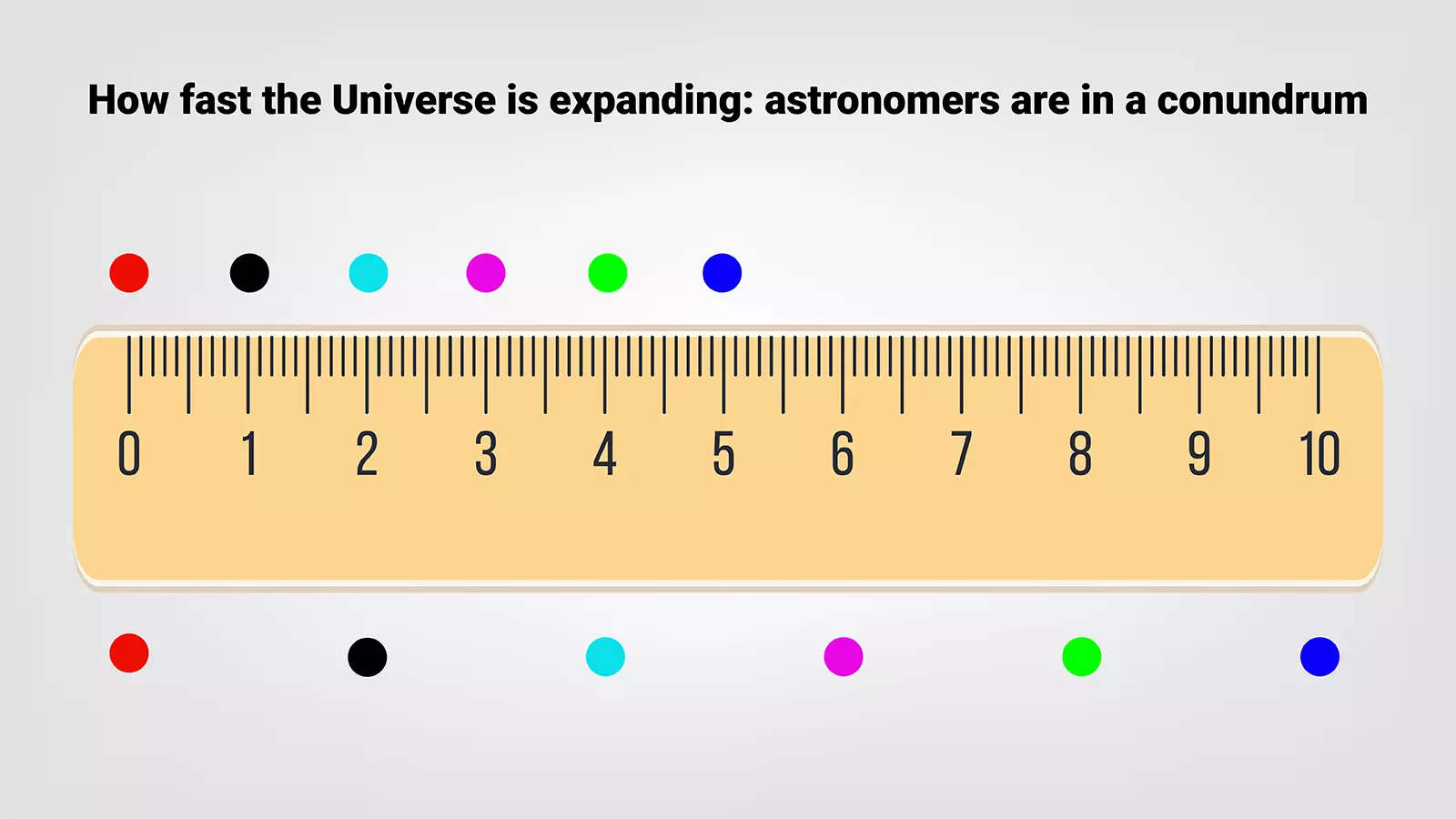
When we expand the elastic strip, the relative distance between the dots increase.
The stretched string is twice as long as the original. As a result, the first dot on the stretched string would have shifted two centimetres away from the hook. The second dot would have travelled twice its initial distance, resulting in a four-centimetre distance from the hook in the stretched string. Likewise, in the stretched string, the third dot will be at 6 cm, the fourth at 8 cm, and so on. This implies that the first dot moved one centimetre from its previous place, the second two centimetres, the third three centimetres, and so on.
Assume that the string is stretched to twice its length in one second. This implies the second dot moved two cm in one second, but the first dot moved one cm in a second. Thus, the second dot had to have travelled more quickly to reach its place in the stretched string than the first dot. The third dot travelled 3 cm in a second; hence, its speed had to be greater than that of the second one. You must have moved faster the farther you are in the string.
From the Hubble telescope to the James Webb Space Telescope, the telltale evidence of the Universe's expansion is unmistakable in whichever direction we look.
How to measure the Hubble constant
To compute the Hubble constant from observational data, we need to know how far a cosmic object is and how fast it is receding from us.
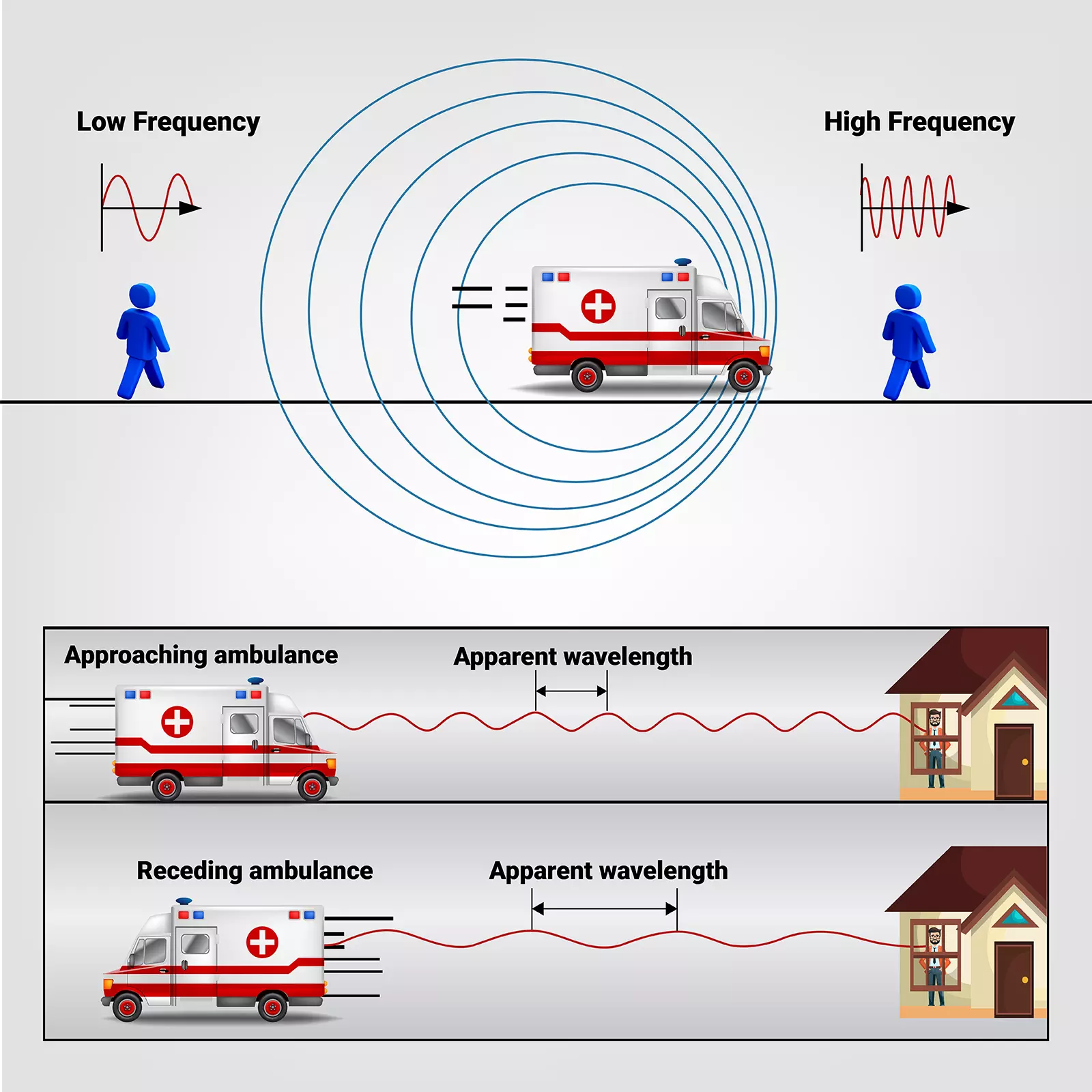
As the source approach, the wavelength becomes shorter, and when the source recedes, the wavelength becomes longer.
Measuring the speed at which a celestial object moves away is straightforward using the Doppler effect. The sound waves from the siren of an approaching ambulance are compressed and stretched as it races away, which we observe as a change in the siren's pitch. This effect, called the Doppler effect, also applies to light. The wavelength of light from distant galaxies moving away from the Earth is stretched like the siren sound waves from the receding ambulance. This stretching of the waves is what astronomers call ‘redshift’. In the spectra of the stars or galaxies receding from us, the spectral lines shift towards the higher wavelengths, that is, towards the red end of the spectrum. The greater the redshift, the faster the object moves away from us.
Finding the distance of a deep-space stellar object using the 'standard candle method' is a bit more complicated, but the principle is simple.
Let us say a 60W bulb dimly shimmering on a distant lamppost. The luminance of the bulb is brighter or dimmer depending on the distance. The brightness of a light source diminishes as the square of the distance. If the distance of the 60 W bulb doubles, the intensity will reduce by 1/4. We know how bright a 60 W bulb should shine. By comparing how faint it appears at a distance, we can compute the distance using the inverse square relationship between brightness and distance.
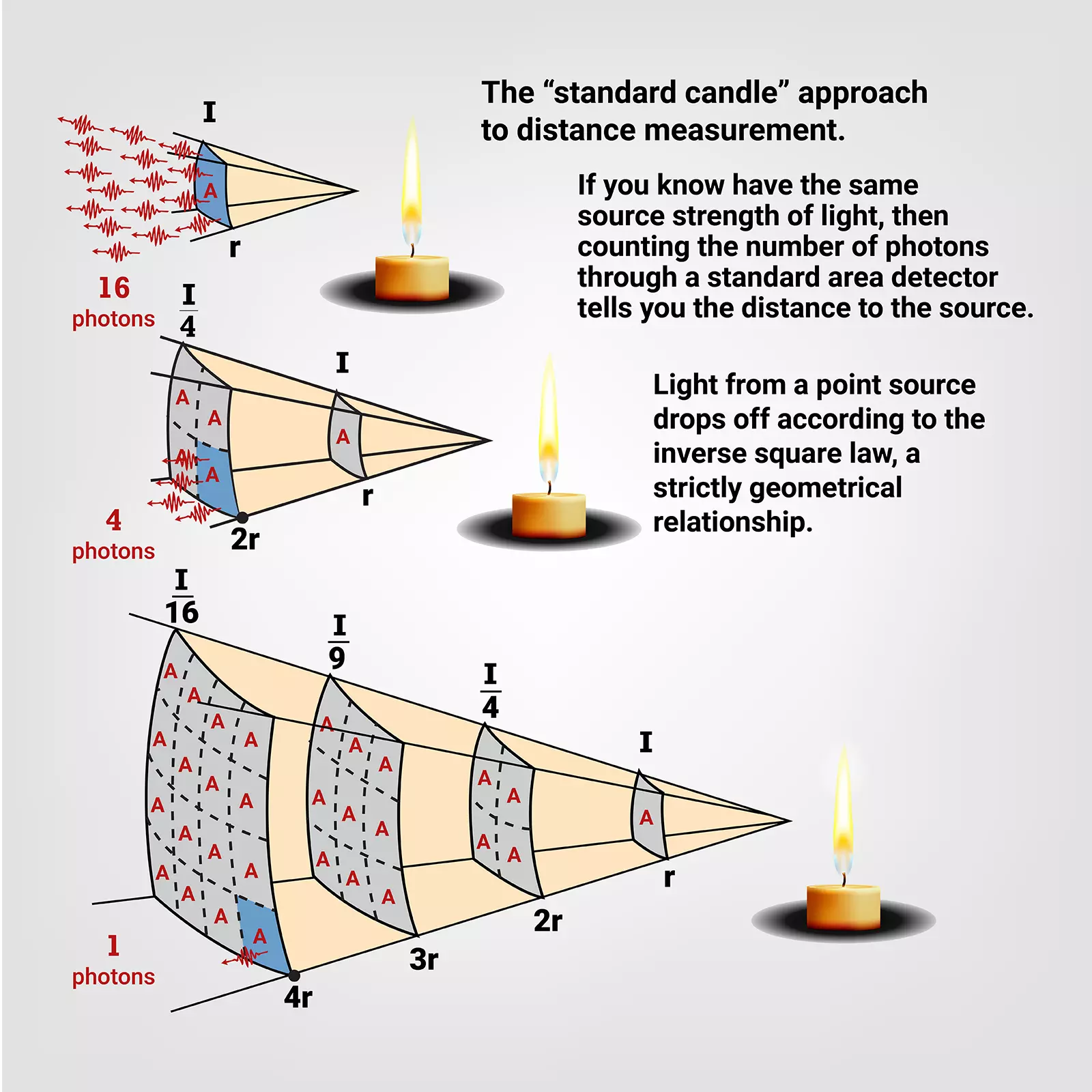
The 'standard candle' approach
We can use a similar strategy to find the distance of stars and galaxies. We know the intrinsic brightness of particular stellar objects, such as flashing stars, called Cepheid variables, and a unique class of stellar explosions called Type Ia supernova. These celestial objects are called 'standard candles'. Once we detect a 'standard candle' in a distant galaxy, we can precisely observe its apparent brightness and compare it with its known intrinsic brightness. We can estimate the distance to the 'standard candle' and the cluster of stars hosting this star using the inverse square law.
The Hubble Space Telescope and James Webb Space Telescope, two of the most powerful tools in astronomy, have observed thousands of Cepheid variables and hundreds of Type Ia supernovae. These observations have been crucial in estimating the Hubble constant, a key parameter in our understanding of the Universe's expansion. The current estimate of the Hubble constant from these observations is in the range of 73-74 km/s/Mpc.
From darkness to light
All galaxies are retreating from one another. Rewinding the process, we discover that about 13.8 billion years ago, all objects in the cosmos were confined to a relatively small region, a fraction of the point. In what is known as the Big Bang, the cosmos expanded to around 10.6 light-years (100 trillion kilometres) in a minuscule fraction of a second. The Universe kept expanding since then. This is the Big Bang cosmology in a nutshell.
Initially, the Universe was just made up of energy; even quarks, the fundamental constituents of matter, were produced only after 0.01 milliseconds. Neutrons and protons emerged about 100 seconds after the Big Bang when the cosmos was roughly 100 light-years across and thousands of times smaller than the Milky Way galaxy.
As the Universe expanded, the temperature decreased. After three minutes of the Big Bang, the temperature dropped from approximately 10^32 degrees (one followed by thirty-two zeros) to a few thousand million degrees. However, the temperature was still too high for the atomic nucleus of hydrogen, the proton, to hold an electron attached to itself. Free-roaming electrons joined the proton to create the hydrogen atom for a fleeting instant. However, the electrons were quickly yanked by a collision with a fast-moving photon. The freely flowing electrons interacted with the photons (light 'particles'); as a result, the light got stuck and could only go a short distance before striking an electron. The Universe was dark.
When the Universe was roughly 3,000,000 years old, it was 1000 times smaller than today. By then, the expanding cosmos had cooled to approximately 3000 degrees, which allowed electrons to stay bonded to the nucleus. During this epoch, the first stable hydrogen and helium atoms emerged. The photons could now traverse longer distances. The Universe turned transparent. The first glow of the Universe raced towards every direction.
As the cosmos expanded thousands of times to its current size, the wavelength of these relic radiations expanded to microwaves. The residue of this old, initial glow can be observed in all directions of the sky as Cosmic Microwave Radiation (CMB).
The CMB is the farthest and oldest light, bringing astronomers as close as possible to the Big Bang. It is impossible to see beyond this point because the Universe was dark before this era.
Clues from Cosmic Microwave Background Radiation
Up to 3,000,000 years from the Big Bang, the cosmos was a soup of virtually uniform, dense, hot plasma comprised of electrons, protons, and light. However, there were minor fluctuations, only one part in 100,000. Nonetheless, even these little weeny clusters possessed greater mass and, hence, relatively higher gravity, resulting in more mass collecting at the place. However, the temperature was so high, and the particles travelled so fast that they clashed and broke apart. Pressure waves propagated across the plasma as gravity's pulling force alternated with the collision's repelling impact.
Ordinary sound waves are simply travelling compressions and rarefactions of gas, which we perceive as sound when they impact our eardrum. The compressions and rarefactions move through the plasma as compression waves, similar to sound waves. Compression causes clumpy matter that is heated compared to rarefaction.
When the Universe was about 3,00,000 years old, it cooled to roughly 3,000 degrees, allowing stable atoms and neutral molecules to form. Gravity now gained the upper hand, and pressure wave propagation came to a halt. As a result, matter did not move from its location, and regions with higher density than average remained as is. Around this time, small-scale ripples were carved into the distribution of matter and energy across the Universe in the same way that a frozen lake clings onto the overlapping crests of waves.
The early Universe's denser matter patches evolved into regions with a higher density of galaxies and galactic clusters. The echo from the early cosmos, these cosmic vibrations, can be used to estimate the Hubble constant.
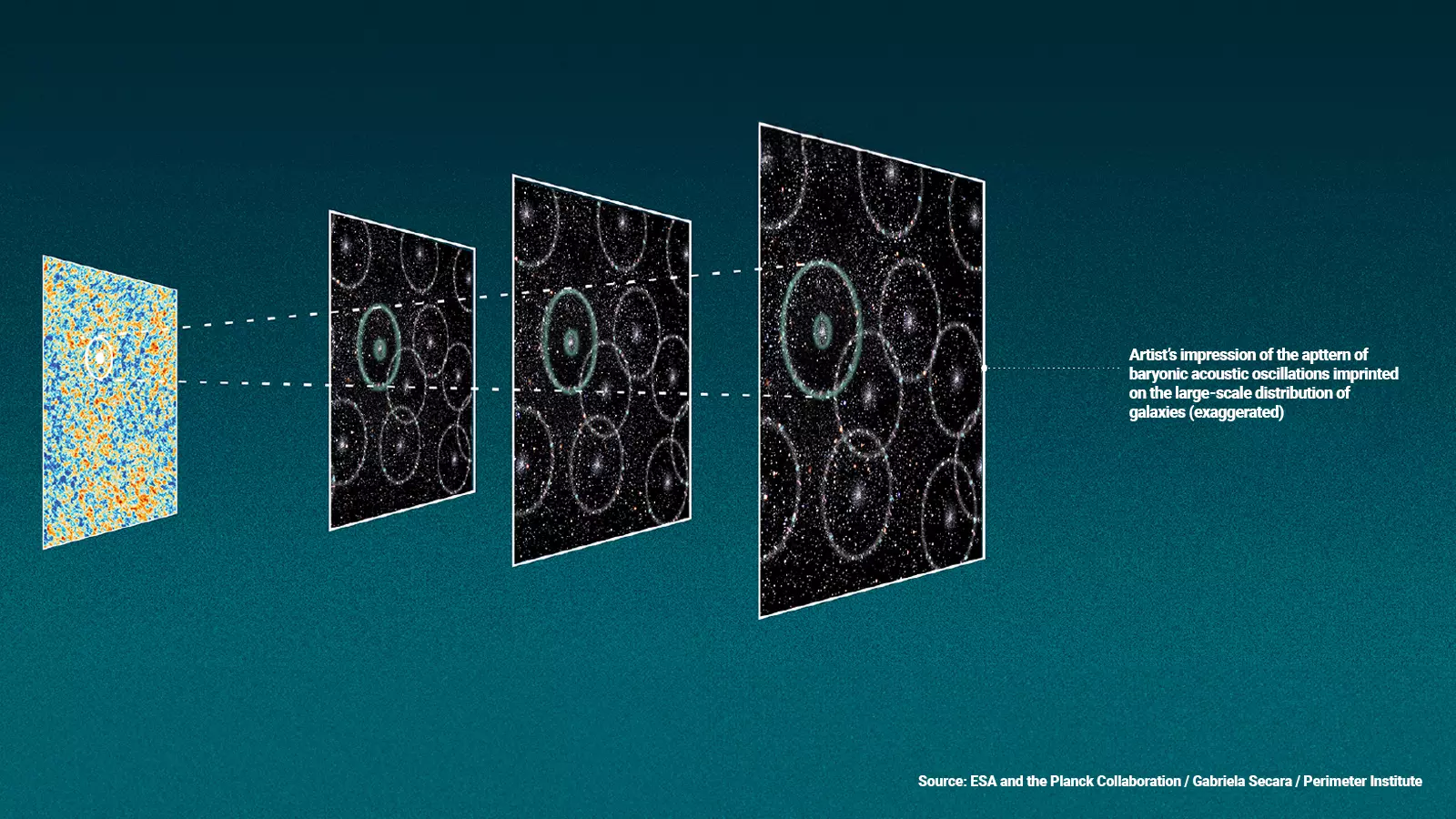
The tiny ripples where matter aggregated at around 3,00,000 years after the Big Bang are the foundations on which the present large scale structure of the Universe is built. By measuring each bubble, the Sound Horizon, we can arrive at the Hubble Constant through an alternate method. Photo: Chris Blake and Sam Moorfield (ESA and the Planck Collaboration / Gabriela Secara / Perimeter Institute)
Over the past 13.7 billion years, the cosmic background radiation has cooled to 2.7255 K or minus 270.4245 Celsius. This radiation is visible uniformly from all directions. Nonetheless, tiny variations of only tens or hundreds of micro-degrees are discernible in the otherwise uniform cosmic microwave background radiation corresponding to the clumps and voids in the early Universe.
The relatively clumpy areas evolved into stars, galaxies, and galactic clusters, with rarefactions becoming massive cosmic voids. We can estimate the amount of time elapsed by comparing the distribution of clumps to the current dispersion of galaxy clusters and large-scale structures. Cosmologists can calculate the Hubble constant by comparing the measured angular size of the sound horizon, clumping, or hot spots in the CMB to their known physical dimensions. This permits astronomers to determine the Hubble constant using a different method.
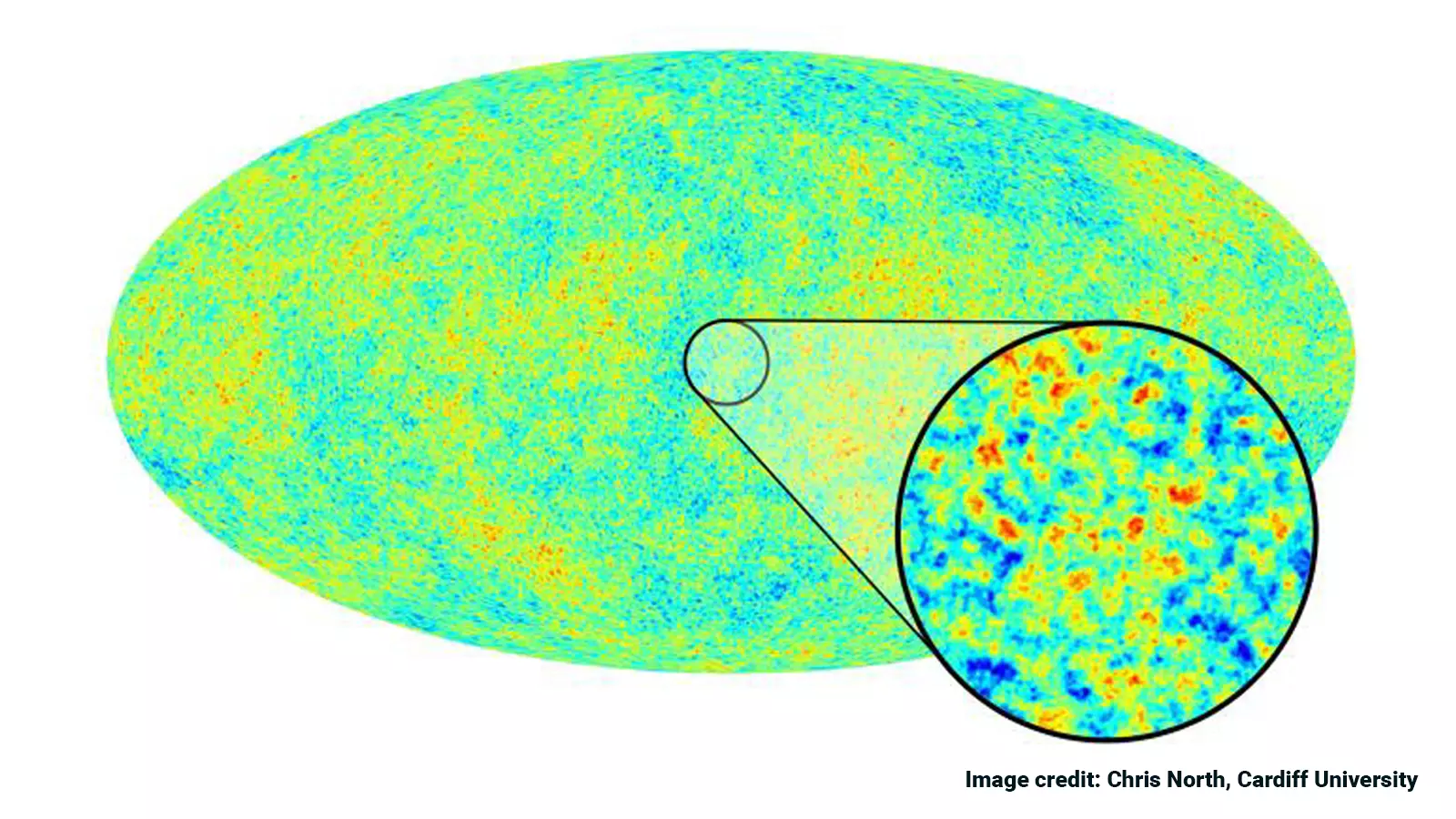
The tiny fluctuations in the temperature shown in false colour are clumps and voids in the early universe, which became the seeds for the formation of galaxies and large scale structure of the present universe.
Space observatories, rather than those on Earth, can detect CMB radiation through precession. One of the first was the Soviet RELIKT-1, which flew on the Prognoz 9 satellite launched in 1983. It measured the uniformity of radiation in all directions. Following that, the NASA Cosmic Background Explorer (COBE) spacecraft orbited the Earth from 1989 to 1996. These missions were incapable of detecting minute variations. NASA's Wilkinson Microwave Anisotropy Probe, launched in 1991, and the European Space Agency's (ESA) Planck Surveyor, launched in May 2009, gave high-resolution CMB images that clearly showed the fluctuations. Using the CMB measurements, cosmologists calculate the Hubble constant to be 67-68 km/s/Mpc with high precision.
Towards a new physics?
Two rigorous methods of computing the value of the Hubble constant differ significantly. Astronomers thought the launch of the James Webb Space Telescope and the new cycle of Planck measurements would bring about agreement. Sadly, the chasm is only increasing.
There must be something fundamentally wrong with one of the methods for measuring the Hubble constant, or there must be physics that we do not yet know. We have found no errors in the Planck measurement of the Hubble constant or the JWST observations. Perhaps the conflict between the observations indicates that our current model of cosmology is flawed or that new physics is required. The jury is still out.
J.B.S. Haldane, a British-born Indian evolutionary biologist, famously stated that "the universe is not only stranger than we imagine, but stranger than we CAN imagine." Who knows what is in store?
(The story first appeared on The Federal on August 14)
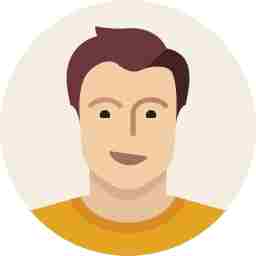